BACKGROUND
Environmental effects on epigenetics are probably more important during the prenatal and early postnatal development, when epigenetic mechanisms are established. Error accumulation during epigenetic information maintenance contributes to inter-individual variation with age (1,2).
Nutriepigenomics (the study of epigenetic modifications including the interaction between nutrients and DNA) with an appropriate nutritional management through healthy, balanced, and personalized nutrition can prevent certain diseases and related complications (3).
Maternal nutrition around the time of conception and during gestation determines proper fetal development and reflects on the long-term health of the offspring. Indeed, placental development and function heavily depend on the mother’s diet (4). In addition to maternal diet, the diet consumed by the offspring during the post-weaning period also influences their health in later life significantly (5).
Folic acid is one of the vitamins that women consume the most during gestation. Folate, the synthetic form of this vitamin, participates in one-carbon (1C) metabolism. 1C metabolism involves a network of interrelated biochemical reactions that include the methionine and folate cycles. These cycles are central to cell function and provide 1C units (methyl groups) for nucleotide biosynthesis, which in turn are essential for DNA and RNA synthesis. Folate is a generic term for compounds with activity that resemble the activity of pteroylglutamic acid, a water-soluble vitamin that has tetrahydrofolic acid (THF) as the biologically active form and therefore enters the folate cycle. THF is then converted to 5,10-methylene-tetrahydrofolate (5,10-CH2-THF) and irreversibly reduced to 5-mTHF. Alternatively, 5-mTHF can be converted to 10-formyl-tetrahydrofolate (10-f-THF) through a series of reactions catalyzed by methylenetetrahydrofolate dehydrogenases. 5-mTHF demethylation completes the folate cycle as 1C is donated for homocysteine (HCY) remethylation to methionine (6,7).
Nutrients such as folic acid, vitamins B12 and B6, riboflavin, choline, betaine, and methionine have crucial roles in this biochemical pathway (8,9). Micronutrient deficiency or supplementation can disrupt 1C metabolism and directly affect genome integrity through inadequate uracil insertions into DNA, which changes DNA methylation and gene expression patterns (10).
Folic acid intake influences DNA methylation patterns and gene expression, and takes part in mechanisms that potentially impact chronic disease development. Therefore, adequate folic acid consumption helps organisms to develop healthily (11). According to the Dietary Reference Intakes (DRI) (12), the recommended folic acid intake by adults and pregnant women is 400 and 600 µg/day, respectively.
Animal studies have shown that maternal folic acid and vitamin B12 deficiency or supplementation in the peri-conceptional period may influence the establishment of DNA methylation patterns and thus alter gene expression and phenotype in the offspring (13,14). Huot et al. (15) showed that offspring that consumed the same diet supplemented with folic acid during gestation and post-weaning could have altered gene expression.
Environmental factors, like diet, physical activity, stress, and exposure to alcohol and tobacco during gestation can greatly impact the health of the offspring through changes in epigenetic mechanisms such as DNA methylation and histone modification. Therefore, environmental factors may play a critical part in determining the risk of an individual developing metabolic diseases during adulthood, including heart disease, allergies, neurodegenerative diseases, obesity, and some cancers (16,17).
Investigations into obesity have identified some genes that are involved in this disease, like the proopiomelanocortin (Pomc, an anorectic peptide) gene (18). The Pomc gene is expressed in the arcuate nucleus of the hypothalamus, in the anterior and intermediate lobes of the pituitary gland, as well as in several other tissues such as the placenta, gastrointestinal tract, reproductive tract, lungs, and lymphocytes (19,20). This gene has been mapped to chromosome 2p23.3 and 6q14 in humans and mice, respectively (21,22).
Pomc deficiency in the arcuate nucleus of the hypothalamus increases food intake and reduces energy expenditure, which culminates in obesity and metabolic and endocrine disorders. Zhan et al. (23) verified that Pomc neurons have different functions in the arcuate nucleus of the hypothalamus and in the nucleus of the solitary tract, which suggests that these neurons regulate energy feeding and homeostasis by integrating long-term hypothalamus adiposity signs and short-term brainstem satiety signs.
In humans, the first evidence that melanocortins (originating in Pomc proteolytic cleavage) participate in food intake control emerged from the description of two patients who developed very early obesity, and who had mutations in the Pomc gene. Similarly, Pomc-knockout mice were obese and hyperphagic, and had impaired pigmentation and adrenal gland function (24).
Because the Pomc gene bears a promoter region, it is rich in CpG islands and is methylated. These features are relevant during experiments involving folic acid manipulation: this vitamin takes part in 1C metabolism, enabling reactions with methyl groups and consequently altering gene expression.
The present study aims to investigate Pomc gene and protein expression in female rat offspring subjected to a folic acid-deficient diet.
METHODS
This study followed the Ethical Principles on Animal Experimentation and was approved by the National Council of Animal Experimentation Control (CONCEA) of Ribeirão Preto Medical School, University of São Paulo, under protocol number 005/2014-1.
Wistar rats obtained from the University of São Paulo Central Animal Housing Facility at Ribeirão Preto were used in this research. At the Animal Housing Facility, Department of Medical Clinics, Ribeirão Preto Medical School, University of São Paulo, the rats remained in individual plastic boxes (width = 44 cm, length = 50 cm, and height = 27 cm) at controlled temperature (22 °C) and under a 12-hour dark/light cycle with food and water ad libitum. Throughout the treatment, rat weight and food intake were measured three times a week. First, the rats received commercial food for three days, which allowed them to adjust to the new site. Then, they were fed the modified diets (Table I), which were based on AIN 93G (American Institute of Nutrition) (25). To this end, the rats were separated into two distinct treatment groups: the control group (2.0 mg of folic acid/kg of food; two females and one male) and the folic acid-deficient group (0.5 mg of folic acid/kg of food; two females and one male). Within the corresponding group, the rats remained together for mating for approximately one week. They were separated after pregnancy was confirmed.
During gestation and lactation, which lasted a total of approximately 42 days (21 days for each period), the mothers remained on the modified diets. After weaning, the offspring also started being fed the modified diet corresponding to their mothers’ treatment group for three months. Figure 1 depicts the treatment protocol.

Figure 1. Protocol for female offspring treatment during the study (FC: female offspring in the control diet group; FD: female offspring in the folic acid-deficient diet group).
A total of 10 female offspring, five per treatment group, were analyzed (n = 5/group). After treatment, all the female offspring were euthanized with 300 mg of ketamine/kg and 30 mg of xylazine/kg and decapitated. The rat brain was removed. All the procedures abode by the guidelines of the Ethics Committee on Animal Experimentation of Ribeirão Preto Medical School, University of São Paulo. The brain was weighed on an analytical balance (Mettler®, Ae200), wrapped in autoclaved aluminum foil, and immediately frozen in dry ice and stored in a freezer at -80 °C.
The arcuate nucleus (ARC) of the hypothalamus was extracted at the Laboratory of Histology, Department of Physiology, Ribeirão Preto Medical School, University of São Paulo.
To perform this step, the cryostat (Microm International/HM 500 OM) was used so that the brain tissue was manually sliced at low temperature (-18 °C) and the ARC sample mRNA was not degraded. To localize the ARC correctly, reference points in the Atlas Brain Maps, Structure of the Rat Brain (26), were employed. The frozen brains were initially cut into 30 µm coronal sections. The tissue was cut until the hippocampus became visible and the optic tract became smaller and began to lateralize, which left the lower part of the brain irregular. Thereafter, a 1500 µm-thick cut containing the ARC was made. This slice was then transferred to a pre-cooled sterile glass slide where the ARC was withdrawn with a 1.5 mm autoclaved punch needle. The ARC was placed in a 1.5 mL sterile microtube containing RNAlater® Solution (Ambion®, Foster City, CA, USA) and stored in a freezer at -80 °C until it was processed.
RNA was extracted from the collected tissue with the TRIzol® Reagent (Life Technologies™, Carlsbad, CA, USA) according to the manufacturer’s protocol. The extracted RNA was quantified on the Nanodrop 2000 apparatus (Thermo Fisher Scientific, Waltham, MA, USA), and its purity was evaluated by A260/A280 (1.8-2.0) and A260/A230 (2.0-2.2) ratios. The RNA was then treated with the enzyme RQ1 RNase-Free DNase® (Promega™, Fitchburg, USA) according to the manufacturer’s protocol. The amount of treated RNA was 1500 ng. The reaction was carried out in the Thermal Cycler Veriti 96-well Thermal Cycler (Applied Biosystem®, Foster City, CA, USA). cDNA was synthesized according to the SuperScript® VILO™ MasterMix (Life Technologies Inc., Carlsbad, CA, USA) manufacturer’s protocol. The reaction was conducted in the Thermal Cycler Veriti® 96-well Thermal Cycler (Appied Biosystem, Foster City, CA, USA). The following cycle was accomplished: incubation at 25 °C for 10 min, incubation at 42 °C for 60 min, and incubation at 85 °C for 5 min, when the reaction was terminated.
The relative Pomc gene expression (Pomc-Rn00595020_m1) was quantified by real-time PCR in a 7500 Fast Thermal Cycler apparatus (Applied Biosystem®, Foster City, CA, USA). TaqMan® Array Fast Plates (Life Technologies Inc., Carlsbad, CA, USA) were used, and the manufacturer’s protocol was followed. The samples were analyzed by the 2-ΔΔCt method(27), in triplicate; the Expression Suite Software v1.0.3 (Thermo Scientific™) was employed. Beta-actin (Actb-Rn00667869_m1) and hypoxanthine phosphoribosyltransferase-1 (Hprt1-Rn01527840_m1) were used as the reference genes. The appropriate cDNA concentration was 50 ng for a final reaction volume of 20 µL.
The protein was extracted by using a modification of the TRIzol® Reagent (Life Technologies Inc., Carlsbad, CA, USA) protocol. The protein was re-suspended in 100 µL of 1% SDS (sodium dodecyl sulfate). To solubilize the protein completely, the sonicator Virtis Virsonic 100 Ultrasonic Cell Disruptor (five cycles that included 15 sec in the sonicator and 30 sec of incubation on ice) was employed. Then, the samples were taken to a water bath (ALB 800s; INBRAS™) at 100 °C for 3 min and centrifuged at 8,700 rpm and 4 °C for 10 min.
The protein was quantified in each sample by means of the Pierce™ BCA Protein Assay Kit (Thermo Scientific™, Rockford, USA) protocol. Absorbance at 562 nm was read on the spectrophotometer SpectraMax® M3 (Molecular Devices, Sunnyvale, CA, USA) after the samples had been incubated at 37 °C for 30 min.
For electrophoresis, 4-15% Mini-PROTEAN® TGX™ Precast Protein Gels (Bio-Rad, Hercules, CA, USA) were used. Tris-Glycine-SDS was the run buffer for protein separation. The molecular weight marker PageRuler™ Prestained Protein Ladder (Thermo Scientific™, 5 µL) was added to the first well of the gel, whereas 20 µL of the sample containing the protein extract (3 µg of protein per well) was added to each of the other wells. The race started at 80 V for 10 min. The voltage was increased to 100 V and maintained at this value for 1 h 20 min.
Subsequently, the gel was transferred to a TransBlot® Turbo™ Mini Nitrocellulose Transfer Packs (Bio-Rad, Hercules, CA, USA) nitrocellulose membrane with the Trans-Blot® Turbo™ (Bio-Rad, Hercules, CA, USA).
After being blocked with 5 mL of 5% BSA in TBS for 1 h, the membrane was incubated with the primary antibodies at 4 °C overnight (16 h). The primary antibodies were as follows: 1 µL of anti-β-actin (mouse, monoclonal, 1:10,000, Sigma 5441) for the endogenous antibody, and 6.25 µL of anti-POMC (Rabbit, polyclonal, 1:800, ab94446) for the reference gene. Next, the membrane was washed with TBS-T, placed in 5 mL of 5% BSA and 1 µL of anti-mouse IgG HRP conjugated secondary antibody (Goat, 1:5000, sc2302) for the endogenous antibody (anti-β-actin), and incubated for 1 h. For the reference antibody (anti-POMC), 1 µL of anti-Rabbit IgG HRP conjugated secondary antibody (Goat, 1:10,000, ab97051) in 10 mL of 5% BSA was used for 1 h. Soon after that, the membranes were washed with TBS-T, and the Amersham™ ECL™ Select Western Blotting Detection Reagent (GE Healthcare Life Sciences, Marlborough, MA, USA) developer was added for analysis on an ImageQuant LAS 4000 (GE Healthcare Life Sciences, Marlborough, MA, USA).
The final protein quantification, based on the bands, was performed with the Image Studio Lite software v.3.3.1 (LI-COR Biosciences, Lincoln, Nebraska, USA).
Descriptive statistics based on the mean and standard deviation were used. The Shapiro-Wilk test helped to verify data normality. The t-test was used to compare weight and gene and protein expression values. The Mann-Whitney test helped to analyze consumption data. All the statistical analyses were performed with the Statistical Package for Social Science (SPSS version 20); a significance level of 5% (p < 0.05) was adopted.
RESULTS
The body weight and food intake measured for the female offspring in the control diet group and the female offspring in the folic acid-deficient group were not statistically different (p > 0.05; Fig. 2).

Figure 2. Body weight and food intake. A. Body weight of female offspring from 0 to 13 weeks post-weaning, in grams. FC: female offspring in the control diet group; FD: female offspring belonging to the folic acid-deficient diet group. Data represented as mean ± SD (standard deviation), n = 5 per group. The t-test was used. B. Food intake of female offspring from 0 to 13 weeks post-weaning, in grams. FC: female offspring in the control diet group; FD: female offspring in the folic acid-deficient diet group). Data represented as mean ± SD (standard deviation), n = 5 per group. The Mann-Whitney test was used.
The Pomc gene mRNA expression in the ARC of the female offspring in the folic acid-deficient diet group was significantly higher than the Pomc gene mRNA expression in the ARC of the female offspring in the control diet group (p = 0.03; Fig. 3).
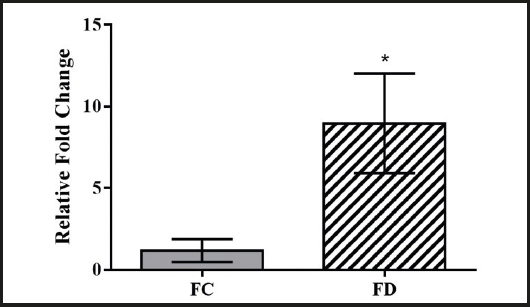
Figure 3. Relative Pomc gene mRNA expression in the arcuate nucleus of the hypothalamus (FC: female offspring belonging to the control diet group; FD: female offspring belonging to the folic acid-deficient diet group). Data represented as mean and ± SD (standard deviation), n = 5 per group. *p = 0.03. The t-test was used.
The Pomc protein expression in the ARC of the female offspring in the folic acid-deficient diet group was significantly higher than the Pomc protein expression in the ARC of the female offspring in the control diet group (p = 0.01; Fig. 4).
DISCUSSION
The study involved female rat offspring born from mothers receiving a control or folic acid-deficient diet and fed the same diet during post-weaning for thirteen weeks. Samples were collected from the arcuate nucleus of the hypothalamus of the female offspring for Pomc gene and protein expression analyses.
Changing the amount of folic acid (0.5 mg/kg of food) that was consumed during gestation, lactation, and post-weaning significantly altered the Pomc gene and protein expression even though the dietary intake and weight gain did not differ significantly between the offspring in the folic acid-deficient diet group and the control diet group.
Folic acid is a vitamin that plays key roles in many cellular reactions: it participates in purine and pyrimidine biosynthesis (for DNA and RNA synthesis), amino acid metabolism, and primary methylating agent (namely S-adenosyl methionine, or SAM) formation (28).
Given the countless folic acid functions during the embryonic period and because clinical practice has shown that folic acid deficiency is no longer common among individuals(29), we decided not to remove all of this micronutrient from the animal food. The absence of folic acid in the diet of pregnant rats causes congenital defects, such as neural tube defects (NTDs) in the offspring, or may even produce non-viable offspring (30). In fact, Maloney et al.(31) observed that the administration of a folic acid-free diet with low methionine and choline concentrations during gestation resulted in smaller offspring.
Several studies have demonstrated that the amount of folic acid in the maternal diet can modify the expression of various genes in the offspring. Mckay et al.(32) conducted a nutrigenomics investigation of liver samples obtained from adult offspring born from mothers that had been subjected to a folic acid-deficient diet (0.4 mg/kg of food). These authors identified 1,859 differentially expressed genes, 920 of which showed increased gene expression.
In contrast, Bermingham et al. (33)analyzed gene expression in colon samples obtained from female offspring subjected to a diet containing selenium and folate (0.6 and 1.8 mg/kg of food, respectively) and verified that 23 genes had higher expression (p < 0.01) during post-weaning. It is noteworthy that Bermingham et al. (33) used a 3.6 times greater amount of folic acid as compared to the present study. These authors also noted that this same diet elevated Dmbx1, Errfi1, Plce1, and Fpr-rs2 gene mRNA expression in liver tissue. However, they did not find any correlations between gene and protein expression in hepatic tissue samples. On the other hand, here we observed a correlation between Pomc gene and protein expression in brain tissue samples.
Diets deficient in vitamin B (folic acid and vitamin B6) influence gene expression in the central nervous system. Herein, a folic acid-deficient diet raised Pomc gene and protein expression in the female rat offspring brain tissue. Almeida et al. (34) treated Wistar rats with vitamin B6-deficient diet during gestation and lactation, and detected significantly higher glutamate decarboxylase-1 (Gad1) gene and protein expression in the offspring hippocampus.
Meher et al. (35) analyzed the hepatic tissue of male and female Wistar rat offspring exposed to different diets during gestation and lactation, and confirmed an altered mRNA expression of hepatic transcription factors. The offspring that received vitamin B12-deficient diet supplemented with omega-3 fatty acids presented a significantly greater PPARα expression, whereas the offspring that received folic acid- and vitamin B12-deficient diet supplemented with omega-3s exhibited a significantly higher PPARγ expression.
Cho et al. (36) reported that folic acid supplementation during pregnancy and after weaning increased Pomc mRNA expression in the hypothalamus of male offspring. These data resembled the results obtained in another study by Cho et al. (37), in which male offspring born from rats that received a multivitamin-rich diet (10 times the amount recommended by AIN-93G) containing folic acid during pregnancy and weaned with the same diet also displayed increased Pomc gene and protein expression in the hypothalamus.
Nevertheless, folic acid supplementation or deficiency may afford divergent results regarding the Pomc gene in male and female rats. Huot et al. (15) verified discrepant results when they fed Sprague-Dawley rat offspring a diet supplemented with folic acid (5 mg/kg of food) during gestation and post-weaning: male offspring on this diet had higher Pomc mRNA expression than male offspring on the control diet, whilst Pomc mRNA expression in female offspring did not depend on the diet. In other words, the same treatment may furnish opposite results in females and males. The mechanisms underlying gender differences upon exposure to a folic acid-deficient diet or folic acid supplementation are not yet clear in the literature.
Gene expression is also a consequence of epigenetic events, which are essential for mammalian development and have a major part in fetal programming(38) and throughout life (4). Some studies have shown that, depending on the amount of folic acid consumed during pregnancy, Pomc mRNA expression may increase and result in lower food consumption and weight gain in the offspring (35 36-37). This happens because the Pomc gene acts as the central regulator of energy homeostasis in the arcuate nucleus of the hypothalamus (39). In hypothalamic neurons, numerous hormones, nutrients, and cytokines regulate Pomc expression. Adipocytes secrete leptin, which mediates Pomc and both cocaine- and amphetamine-regulated transcript (CART) expression regulation (39). Briefly, leptin released by adipocytes stimulates Pomc and CART synthesis, thereby promoting alpha-MSH release by melanocortinergic neurons in the hypothalamus. Alpha-MSH acts on the MC4R and MC3R expressed in these neurons, inhibiting food intake and raising energy expenditure (40). Besides that, leptin also exerts an anorexigenic effect via inhibition of neuropeptide Y (NPY) and agouti-related peptide (AgRP) neurons (41).
Here, offspring belonging to the folic acid-deficient diet group presented higher Pomc gene and protein expression than the offspring belonging to the control diet group, but food consumption and weight in these groups were not statistically different. There are possible explanations for these findings. First, other genes involved in the food consumption cascade may also have had their expression pattern altered, compensating for the increased Pomc expression, as previously explained, and maintaining food consumption and weight unaltered. A second reason for our results may have been the reduced sample size and short intervention period. In contrast, Moloney et al. (31) evaluated 25-week-old female offspring (n = 7) (a relatively longer period as compared to the 21-week-old female offspring assessed here) and found that the folic acid-deficient diet with low choline and methionine concentrations reduced body weight. As for Jadavji et al.,(42) they observed that male offspring (n = 14) born from mothers that received a folic acid-deficient diet (0.3 mg/kg of food), but which did not receive this same diet after weaning, weighed significantly more than the male offspring born from mothers that received a control diet (2 mg of folic acid/kg of food).
Cho et al. (36) revealed that a diet supplemented with folic acid during gestation and post-weaning lowered food intake and body weight by 7% and 9%, respectively, in male offspring (n = 12) at 29 weeks. In another study, these authors also demonstrated that male offspring (n = 13) born from mothers that received a multivitamin diet (10 times the amount recommenced by AIN-93G) containing folic acid during gestation, and weaned with a high-fat diet, had a 5% and 4% lower dietary intake and body weight, respectively(37). In their studies, Cho et al. (36) and Cho et al. (37) provided male offspring with a diet supplemented with folic acid at different periods. However, in both cases, the feeding intervention increased Pomc mRNA expression and decreased food consumption and weight gain.
Although the limitations of the study were a reduced sample size, the specific sex of the animals studied, and that the analyses were performed with only one hypothalamic gene, our results show that environmental factors, such as the presence of smaller folic acid amounts in the maternal diet during gestation and lactation, and in the diet that the offspring was fed during post-weaning, significantly modifies Pomc gene and protein expression, although offspring food consumption and body weight remain unaffected.