INTRODUCTION
Obesity is defined as a condition where abnormal and/or excessive accumulation of adipose tissue leads to the development of an inflammatory condition posing risks to health (1), representing an independent risk factor for a number of metabolic disorders (2). Obesity has a multifactorial etiology that involves environmental, behavioral, psychological and genetic factors (3). An inadequate dietary pattern numbers among these factors, particularly high intake of calories and/or fats, implicated as one of the main determinants for the development of obesity (4).
Chrysobalanus icaco is a medicinal plant popularly known as ‘’Grageru’’ or ‘’Abajeru’’ and characterized as a medium-sized bushy tree found in many regions of Brazil and other Latin American countries, such as Colombia and Venezuela (5,6). Chrysobalanus icaco belongs to the Chrysobalanaceae family. In Brazil, its leaves are used for infusions in folk medicine as an anti-diabetic and anti-lipidemic agent (7,8).
Previous studies have demonstrated the effect of the aqueous extract of Chrysobalanus icaco in the reduction of adiposity and improvement of glucose intolerance (9,10). Analyses of the administration of different doses showed that a dose of 0.35 mg/mL, around 200 mg/kg of bodyweight, produced the best results for improving glucose intolerance and reducing adiposity (10). However, the physiological mechanisms promoting the effect of Chrysobalanus icaco have not been fully elucidated.
Therefore, the objective of the present study was to investigate the mechanism of action of Chrysobalanus icaco in the gene and protein expression of acetyl-CoA carboxylase (ACC), an important enzyme in lipogenesis.
MATERIALS AND METHODS
CHRYSOBALANUS ICACO
An aqueous extract of Chrysobalanus icaco (AECI) leaves was used for this study. The leaves were collected from the village of Jatobá, located within the municipality of Pirambu, Sergipe state. The botanical material was registered under the number ASE 11855 at the herbarium of the Department of Biology, Federal University of Sergipe (UFSE). The extraction was carried out at the Laboratory of Endocrine Physiology and Metabolism of the State University of Ceará (UECE). The dried, macerated Chrysobalanus icaco leaves were submitted to extraction by infusion in distilled water at 100 °C in a proportion of 50 g of leaves per liter of water. The solution thus obtained was left to stand and cool at room temperature (25 °C) for 15 min. Subsequently, the solution was filtered through filter paper, liophylized and then stored at –20 °C for later use.
HIGH-FAT DIET
The control (normocaloric) diet consisted of commercial rat chow (Primor®), whose percentage constituents are: 28 % protein, 60 % carbohydrate, and 12 % fat, yielding 3.24 kcal/g. The high-fat diet, previously standardized, comprised a mixture of high-calorie foods in the following proportions: 1875 g of regular chow, 1250 g of milk chocolate, 1250 g of unsalted skinned peanuts, and 625 g of cornmeal biscuits for every 5 kilograms of feed. These ingredients were ground, mixed, and offered in pellet form. The percentage composition of the high-fat diet was: 14 % protein, 38 % carbohydrate, and 48 % fat. The energy content of the high-fat diet was 4.64 kcal/g.
ANIMALS AND TREATMENT
The aqueous extract was administered at room temperature to animals in the AECI group at an estimated dose of 200 mg/kg/d; it was free from any toxic or other undesired effects (Ribeiro 2020) and was reported to induce glycemic control by Barbosa (2013) and White (2016). During the same period, the vehicle (drinking water) was administered to the other groups (CG and HFD). The doses were adjusted twice a week according to mean weight of the animal. The AECI and vehicle were fractionated and administered twice daily.
During the experimental period, the animals were housed in a temperature-controlled environment (22 ± 2 °C), 3-4 animals per cage, under an artificial 12-h light/12-h dark cycle, and provided with water and chow ad libitum. All experimental procedures were in compliance with the Ethical Principles of Animal Experimentation adopted by the Research Ethics Committee for the Use of Animals of the State University of Ceará, Brazil (number 1847455/2016).
BODY WEIGHT, FOOD INTAKE AND ENERGY EFFICIENCY
Body weight and food intake were assessed twice a week. Daily food intake (FI) was calculated as: diet offered minus leftover food.
Energy efficiency (EE) was calculated by dividing total weight gain (g) by total food intake (kcal), as described by Levin et al. (11).
GLUCOSE TOLERANCE TEST (GTT)
Glucose tolerance was determined at two timepoints: on the 12th week (prior to commencement of treatment with AECI) and after the 16th week (end of experimental protocol). The glycemic curve after oral glucose loading was determined in 12-hour fasted animals after administration of a single dose of glucose (75 mg/100 g bw) by gavage. Glycemia measurements from the tail vein were taken using a glucose meter (One Touch Ultra, Johnson & Johnson’s) at different timepoints: 0 (pre-gavage) and at 5, 15, 30, and 60 minutes after glucose administration. Based on the values from the glycemia curves for each animal, the area under the curve (AUC) was calculated using the software Graph Pad Prism Version 6.0 for Windows.
ADIPOCYTE ISOLATION AND LEPTIN LEVEL
After 16 weeks of the experimental protocol, the animals were killed by guillotine decapitation. Immediately after sacrifice, the retroperitoneal (RP), periepididymal (PE) and subcutaneous (SC) white adipose tissues were collected and stored at -80 °C for later analyses.
Adipocytes were isolated using the collagenase tissue digestion technique, according to Rodbell (12), with some changes to adapt the method to our laboratory conditions. Briefly, the PE adipose fat pads were removed, cut up using scissors into thin fragments, and incubated in 4.0 mL of digestive buffer for around 30 minutes at 37 °C in a bain Marie on an orbital shaker (150 rpm). The sample was then filtered through a plastic sieve with a fine mesh and rinsed three times in 25m L of EHB buffer at 37 °C. Lipids were determined by placing approximately 40 μL of EHB buffer into a glass capillary tube and centrifuging at 2000 rpm for 1 min. The total volume of the suspension corresponds to 100 %, and the volume of adipocytes obtained after centrifugation provides the lipids of the sample.
The serum leptin level was quantified using specific kits for rats RIA (Millipore Corp, St. Charles, MO, USA). All procedures were carried out in accordance with manufacturer instructions.
PROTEIN QUANTIFICATION BY WESTERN BLOTTING
The subcutaneous adipose tissue was weighed and submersed in extraction buffer in Eppendorf tubes. These were homogenized and centrifuged to collect infranatant from the sample, and submitted to polyacrylamide gel electrophoresis until full passage of the proteins through the gel.
The gel was then prepared for the transfer of proteins onto the membrane in an electrophoresis box using transfer buffer with a 0.25-A current for 120 minutes. ACC (Cell Signaling) and α/β-tubulin (Cell Signaling) antibodies were used to analyze the protein expression of these enzymes. The blots were visualized using a bioimaging system (Image QuantTM 400, GE Healthcare), which captured images for 5 to 20 minutes that were then analyzed using the Image Quant Tl (GE Healthcare) software.
GENE EXPRESSION BY qPCR
For gene expression assessment, 100 mg of subcutaneous adipose tissue were homogenized in TRIZOL reagent for RNA extraction. The RNA was purified using a Qiagen kit, according to the manufacturer’s instructions. RNA concentration was analyzed on a Nano Vue device (GE Healthcare Life Sciences) and integrity was assessed using agarose gel. cDNA was obtained by reverse transcriptase reaction on 2 μg of RNA from the sample using the High-Capacity cDNA Reverse Transcription Kit (Thermo Fisher Scientific). A Maxima™ SYBR Greenq PCR Master Mix (Thermo Fisher Scientific) was employed for the qPCR reaction (performed in duplicate). Each reaction comprised 2 μL of sample, 6 μL of master mix, 1.2 μL of primers, and 3.8 μL of water. Gene expression of acetyl–CoA carboxylase was assessed, and beta-actin was used with the constitutive gene. The sequences of the primers used were: ACC (5’- ACAGTGAAGGCTTACGTCTG-3’ and 5’-AGGATCCTTACAACCTCTGC-3’) and beta-actin (5’-TCAGGTCATCACTATCGGAATG-3’ and 5’- TTTCATGGATGCCACAGGATTC-3’).
STATISTICAL ANALYSES
The results were expressed as mean ± SEM. A one-way or two-way ANOVA test, followed by the appropriate post-test, was used for the different comparisons. Differences were considered significant when p < 0.05. Analyses were performed using the GraphPad Prism software version 6.0 for Windows (GraphPad Software, SanDiego, CA, USA).
RESULTS
Comparison of the CG with the HFD and AECI groups revealed a statistical difference in food intake (FI) for total diet consumption in both grams and kcal, where the CG had greater intake for the two parameters assessed. However, no statistical difference between groups was found for energy efficiency (EE) (Table I).
Table I. Body mass, food intake, and energy efficiency for rats fed a standard diet treated with vehicle (CG), a high-fat diet treated with vehicle (HFD), and a high-fat diet treated with aqueous extract of Chrysobalanus icaco (AECI)

Values expressed as mean ± SEM; n = 7-8. *Statistically different from control group, p < 0.05.
Regarding the GTT, prior to treatment, rats fed the HFD had glucose intolerance with a significant increase in fasting glycemia from the first 5 minutes to the end of test, as compared to rats fed the control diet (CG) (Fig. 1A).

Figure 1. Glucose tolerance test (GTT). Glycemia was measured at 0 (baseline), 5, 15, 30 and 60 min after oral administration of glucose. The graphs show the glycemic curve at the time. GTT was performed in two moments: (A) at the 12th week before the start of treatment and (B) at the 16th week at the end of the treatment. *p <0.05; **p <0.01; ***p <0.001. Two-way ANOVA with Bonferroni post hoc test. n = 7-8.
As expected, after treatment, impaired glucose tolerance was observed in the animals fed the high-fat diet, compared to the animals fed the control diet. A similar result is evident in the area under the curve, which showed significant results. The group administered the AECI exhibited improvement in glucose intolerance, although this was not statistically significant (Fig. 1B).
The HFD group had a significant increase (p < 0.05) in PE, RP, and SC adipose mass as compared to the CG and AECI groups (Fig. 2A, 2B and 2C). In the AECI group there was a significant reduction in RP adipose mass to the same mean values measured in animals from the CG (Fig. 2C).
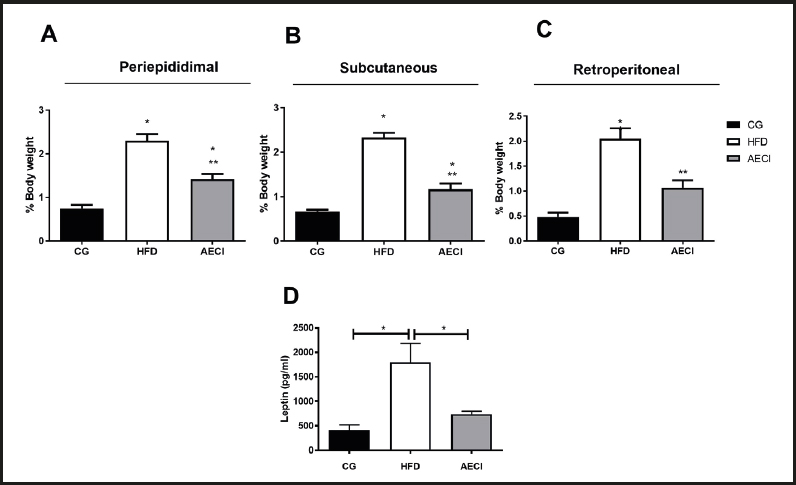
Figure 2. Adipose tissue weight (A), periepididimal (B), subcutaneous (C) retroperitoneal of control groups (CG), hyperlipidic diet (HFD) and hyperlipidic diet and aqueous extract of Crysobalanus icaco trated (AECI). D. Leptin dosage of these groups. *p < 0.05.
With regard to leptin levels, there was a significant leptin increase (p < 0.05) in the animals fed the HFD diet when compared to the animals on the control diet and to rats treated with the extract, where no difference in leptin was evident between these last two groups (Fig. 2D).
The observation of the diameter of adipocytes in PE adipose tissue showed that the HFD significantly increased the size of adipocytes (hypertrophy) relative to the CG. The AECI had a positive effect in reducing the volume of PE adipocytes, reducing them to the same size as the control group, differing statistically from the group fed the HFD without the extract (Fig. 3).

Figure 3. Adipocyte diameter of periepididimal adipose tissue (A) and macroscopic images of tissues of control (B) groups (GC), (C) hyperlipidic diet (HFD) and (D) hyperlipidic diet and and aqueous extract of Crysobalanus icaco trated (AECI).
As depicted in figure 4, the gene expression of ACC was significantly increased (p < 0.05) in the group fed the high-fat diet (Panel A). However, the group fed the high-fat diet and treated with the extract showed a significant reduction (p < 0.05) in the expression of this gene when compared to the HFD group.

Figure 4. Gene expression by PCR (A) and protein by Western Blotting (B), Acetyl-CoA carboxylase (ACC) of subcutaneous tissue of control (GC), hyperlipidic diet (HFD) and hyperlipidic diet and and aqueous extract of Crysobalanus icaco trated (AECI). *p < 0.0.
The animals fed the HFD without extract supplementation showed a significant increase in protein expression of ACC (p < 0.05). The animals in the group supplemented with the extract (AECI) had a significantly lower expression of ACC (p < 0.05) than the group not administered the extract (HFD), although they did not differ significantly from the control group (CG) (Fig. 4B).
DISCUSSION
In the present study we showed that, although not promoting a significant change in intake and weight gain, treatment reduced the weight of SC, RP, and PE fat pads, and also reduced gene and protein expression in the subcutaneous adipose tissue.
Presta and Pereira (13) revealed a hypoglycemic and antioxidant effect of AECI in normal mice using a concentration of 50 mg/mL, although Presta, Fonseca and Bernardo-Filho (14) found that this dose promoted a strong genotoxic effect on plasmid DNA. Akin to the present study, White et al. (15) used a substantially lower concentration (200 mg/kg = 0.0002-0.003 mg/mL) and found this controlled the glycemia of animals in the group treated with the extract. Barbosa et al. (16) also found a significant reduction in glycemia levels in alloxan-induced diabetic rats treated with abajeru extract at doses of 200 mg/kg after six weeks of treatment.
This increase in adipose tissue may be the result of changes in the hypothalamic modulation of the signalling due to the hyperleptinemia induced by the high-fat diet, as seen in the present study. In addition, reduced mitochondrial mass and oxidative capacity has also been observed in rodents fed a high-fat diet, favoring a greater accumulation of fat (17,18).
Some studies involving Chrysobalanus icaco have shown that a diet containing 10 % of seeds from the plant can induce weight reduction in normal rats without toxic effects (19), promote reduction in glycemic levels (9,13,15), and inhibit weight gain and fat in the liver of high-fat diet-induced obese mice treated with the aqueous extract (10); induce analgesic and anti-inflammatory activity using the methanol extract (20); and increase locomotor activity, increasing energy expenditure and preventing fat storage, besides showing an ability to maintain homeostasis of glucose by normalization of insulin sensitivity and glucose tolerance despite a high fat intake (21).
The assessment of the effects of AECI on the adipose tissue of the rats fed a high-fat diet showed lower weight gain in SC, PE, and particularly RP fat pads in the group supplemented with extract, where the difference in RP adipose tissue was not significant as compared to the control group. This reduction in adipose mass was closely correlated with the improvement in glucose tolerance observed in the same group.
In the study by White et al. (21), AECI administered to mice at a concentration of 200 mg/kg promoted a lower gain in adipose mass, possibly explained by a greater energy expenditure caused by the increased locomotor activity induced by the extract, and also by an excretion of fat in the feces, suggesting that the fat from the high-fat diet was not being absorbed in the gastrointestinal tract of the animals treated with the extract. Harach et al. (22) reported that this is a common mechanism of some extracts and compounds isolated from plants for the prevention of obesity.
In the present study, the effects of AECI supplementation on glucose tolerance and reduction of SC, RP, and PE adipose pads might have been due to the level of flavonoids found in this extract. Recently, a study identified the presence of flavonoids derived from myricetin and quercetin in the AECI, including terpenes, aglycone and glycosylated flavonoids (23). Therefore, the beneficial effects on obesity the treatment showed in the present study may be associated with the presence of these metabolites in the extract.
According to Kazemipoor et al. (24), these natural compounds can induce weight loss through several different mechanisms, such as inhibiting pancreatic lipase activity, stimulating thermogenesis, preventing adipocyte differentiation, enhancing fat metabolism, and suppressing appetite. PANG et al. (25) suggested that the decreased intestinal absorption of lipids from the diet results from the effects of saponin through the inhibition of pancreatic lipase enzyme activity. This reduction in lipid absorption promotes a greater mobilization of lipids from visceral adipose tissue to generate energy, thereby reducing the amount of abdominal fat (26).
The homeostasis of adipose tissue is a balance between lipogenesis and lipolysis. The polyphenols present in the AECI appear to lower lipogenesis in adipocytes, and prevent their differentiation by inhibiting the lipogenic enzyme ACC. ACC is a cytosolic enzyme that promotes carboxylation of cytosolic acetyl-CoA into malonyl-CoA, the initial step in the synthesis of fatty acids and important for completing the biosynthesis of triacylglycerol for its incorporation into cytoplasmic fat droplets (27,28).
The gene expression of ACC in the subcutaneous adipose tissue showed that AECI supplementation reduced ACC expression in the group supplemented with the extract. This may explain, at least in part, the reduction in adipose mass seen in the supplemented animals. It is now known that increased lipid synthesis is strongly stimulated by key lipogenic molecules such as SREBP-1c, ACC and SCD-1 (29). This inhibition might have been caused by the action of the flavonoids present in the AECI. Flavonoids are known for having effects such as: antioxidative capacity (30), and anti-inflammatory (31) and hypolipidemic (32) activities that could be revelant to reduce adiposity in obesity models.
According to the results of the present study, the animals treated with AECI exhibited improved glucose tolerance, lower adipose mass gain, and reduced leptin and gene expression of ACC, reducing lipogenesis in the tissues of diet-induced obese rats. These results showed that AECI prevents and/or treats obesity through the action of the flavonoids with biological properties present in Chrysobalanus icaco.